Earth's Stony Legacy – A Solid Foundation
Minerals and rocks have ever since been central to human progress as tools, as construction and raw materials, and as media for art and documentation. Solid rocks constitute the largest volume fraction of planet Earth, including Earth's crust and mantle, providing a solid foundation for life on Earth's surface and for human civilisation. The rocks of Earth's crust and mantle formed as the products of ongoing, dynamic processes and hold records of Earth's past, from the planet's formation until today. Reading this record and projecting Earth's dynamics into the future requires to understand the properties of rocks and minerals as the building blocks of planets.
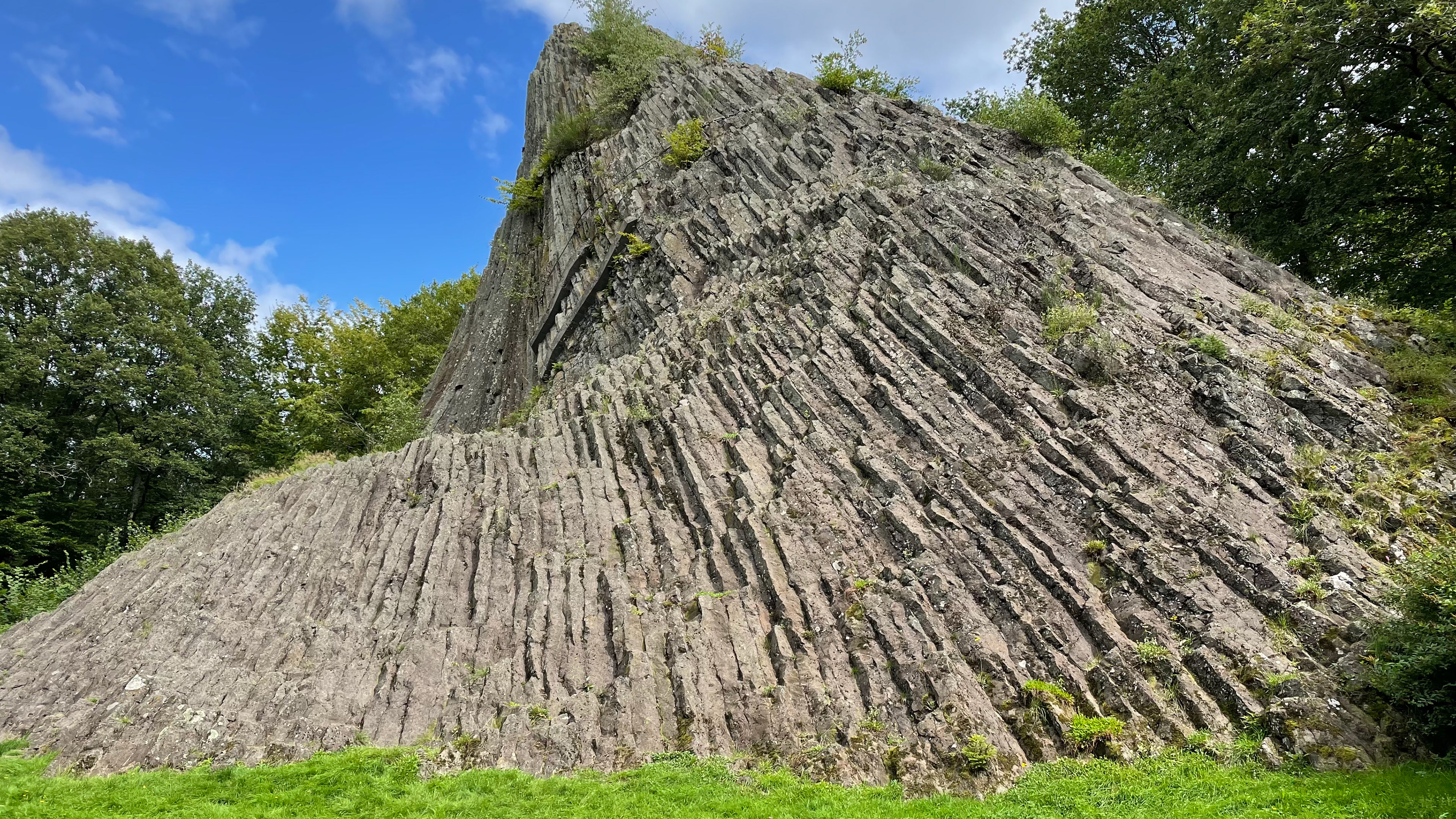
Basalt columns stretch towards the sky to form the cone of the iconic Druidenstein in Kirchen, Germany, EU. The distinctive shape of the basalt cone is believed to have attracted Celtic druids to gather and perform ritual activities in ancient times. In addition to the name Druidenstein (druid stone), the appeal of the rock cone gave rise to local myths and tales. The basalt columns, however, formed about 25 million years ago when basaltic magma intruded into the cold surrounding greywacke rocks and solidified to prismatic columns as a result of cooling and contraction. Over time, weathering and erosion exposed and shaped the cone. Basaltic magma is generated when rocks of Earth's mantle partially melt, for example, beneath regions where Earth's crust is particularly thin, allowing the magma to ascend through the crust. Weathering of basaltic rocks helps stabilise Earth's climate by removing carbon dioxide from the atmosphere. The Druidenstein portrays the connections between Earth's deep interior and surface, between Earth's geologic past and human history, and between rocks and human culture.
Rocks are composed of minerals. The properties of the minerals that form a rock will therefore determine the properties of the rock. Many technologically important materials are also composed of minerals or mineral-like materials such as concrete and ceramics. As with natural rocks, the properties of technological materials, such as mechanical, chemical, and optical properties, will again be inherited from their mineral components.
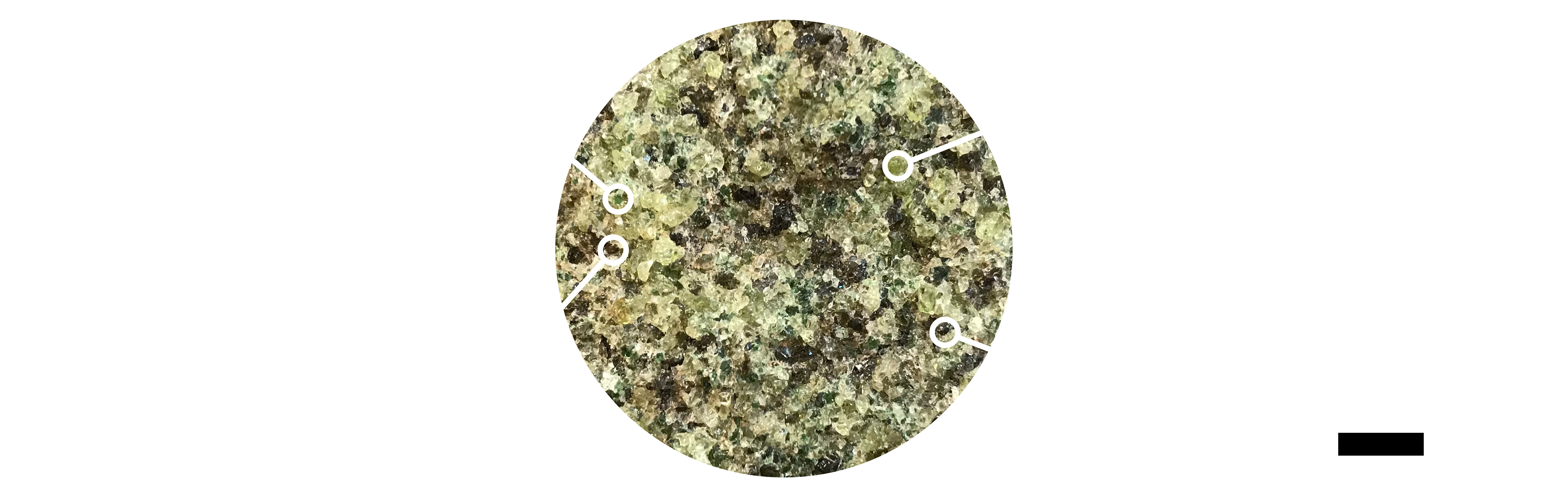
The minerals olivine (pale green), orthopyroxene (dark green/brown), clinopyroxene (vivid green), and less abundant spinel (dark grey/black) form this rock called peridotite, or more specific, lherzolite. Peridotites are believed to be common rocks in Earth's upper mantle because the partial melting of lherzolite generates basaltic magmas that are erupted by volcanoes along mid-ocean ridges and on oceanic islands, such as Iceland, Hawaii, and the Canary Islands. Beneath the sediments on the ocean floors, Earth's crust is composed of predominantly basaltic rocks, emphasizing the importance of basaltic magmatism and peridotites as the associated source rocks in Earth's mantle.
Mineral Physics
My research focuses on the physical properties of minerals. Most minerals form crystals. Crystals are characterised by their periodic arrangement of atoms, ions, and molecular groups in space. It is this three-dimensional atomic order that imparts particular physical properties to crystals. For example, the physical properties of crystals typically depend on the direction of an acting force or perturbation, i.e., crystals show anisotropy (from ancient greek άν-ϊσος-τρόπος for un-equal-direction).
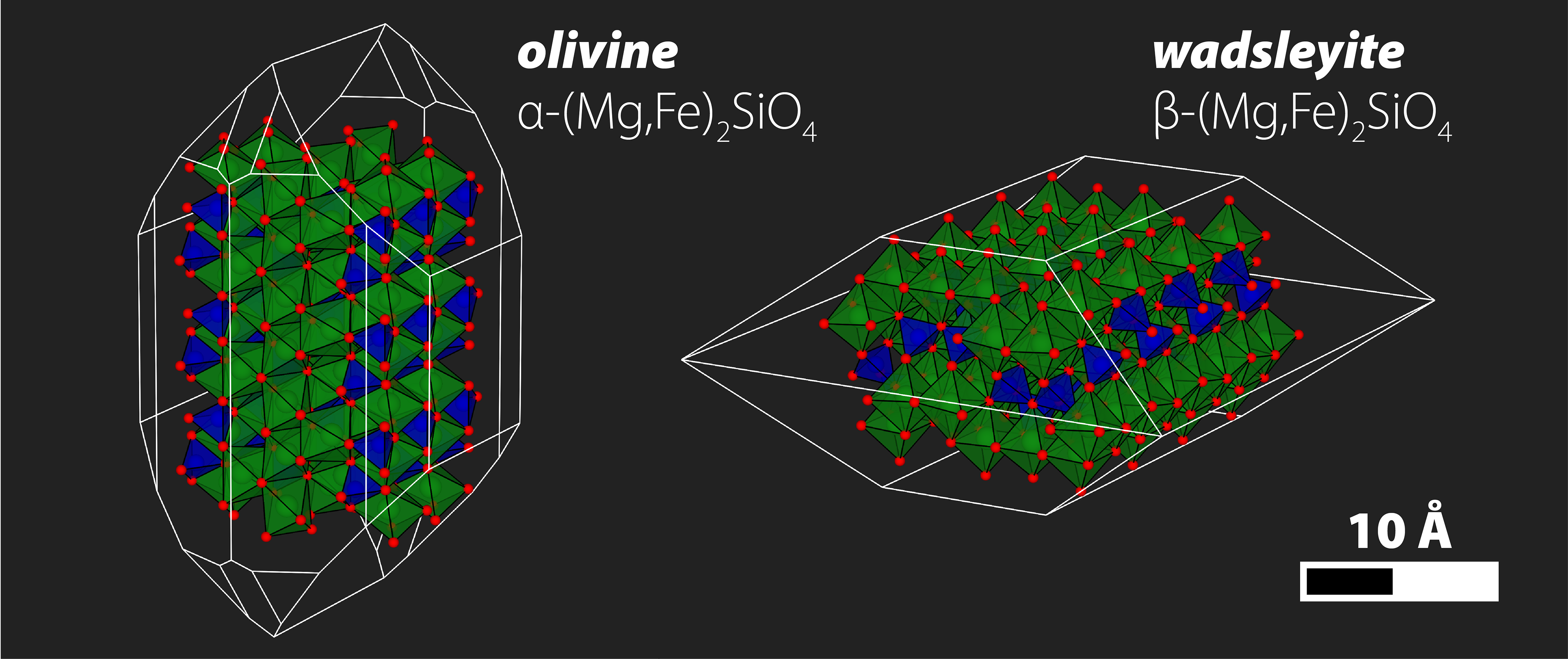
The properties of crystals arise from the combination of different atomic species, i.e., the chemical elements and their ions, and their spatial arrangement into a crystal structure. The graphics above show the crystal structures of the minerals olivine and wadsleyite framed by sketches of typical crystal shapes. Both minerals share the same chemical formula and are composed of magnesium (Mg) and iron (Fe) cations (green), silicon (Si) cations (blue), and oxygen (O) anions (red). The oxygen anions surround the metal cations to form coordination polyhedra (translucent with black outlines). When the mineral olivine is pressurised and heated above certain thresholds, for example, as a component of a rock in Earth's mantle, the atoms rearrange into the crystal structure of the mineral wadsleyite. Both minerals are polymorphs of the same chemical compound but have different physical properties. Graphics were created using the software VESTA (Momma & Izumi, 2011) and crystal structure data from Smyth & Hazen (1973) and Finger et al. (1993). The crystal shape of wadsleyite is inspired by the observations of Shatskiy et al. (2009).
To investigate their physical properties, we irradiate minerals and crystals with electromagnetic waves, such as X-rays, lasers, or infrared radiation, and observe the interaction between a mineral sample and the electromagnetic waves. Depending on their wavelengths, electromagnetic waves interact with minerals by being scattered, diffracted, absorbed, or re-emitted. All these different types of interactions contain information about different mineral-physical properties.
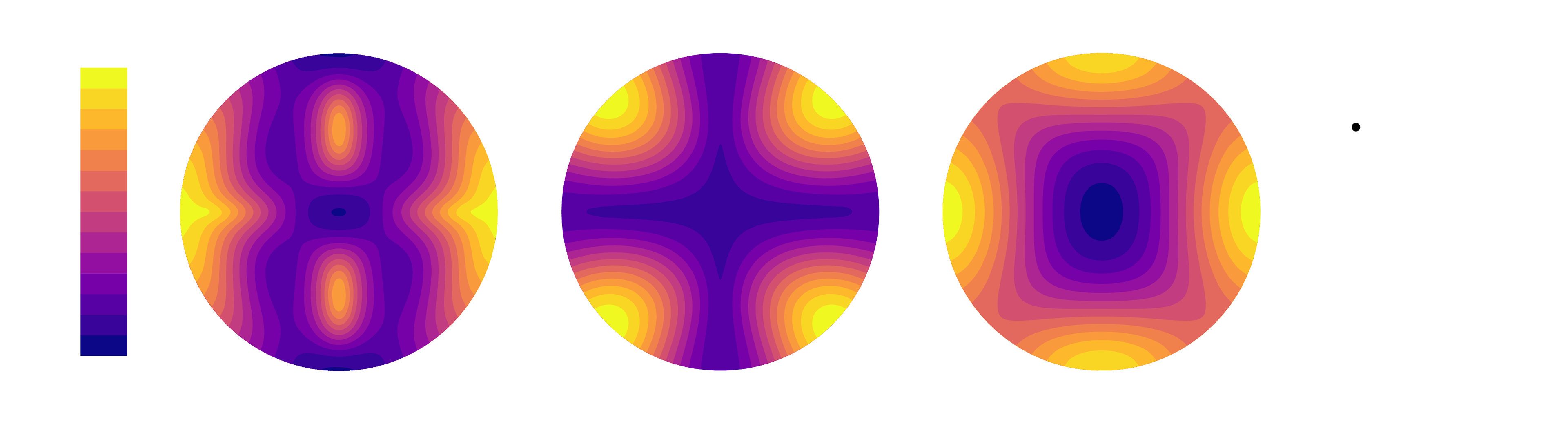
The speed of sound waves that travel through a crystal can be measured by analysing how the light of a laser is being scattered by the thermal motions of the atoms in the crystal structure. In a crystal, there are three types of sound waves for each direction of propagation that are commonly labelled as S1, S2, and P. The diagrams above show how the speed of each type of sound wave varies with the propagation direction of a sound wave in a crystal of the mineral wadsleyite. To read the diagrams, imagine you are looking into the lower half of a sphere. A propagation direction is defined by a line from the centre of the sphere to a point on its surface. In the diagrams above, the propagation directions have been projected onto the plane of the screen, and the associated sound speeds are displayed as colours. The patterns of sound speeds reveal the acoustic anisotropy of a wadsleyite crystal and reflect the crystal symmetry (point group mmm). Diagrams were created using elasticity data from Buchen et al. (2018).
The minerals that form the rocks in Earth's deep interior experience high pressures and high temperatures that change their atomic structure and physical properties. To simulate the extreme conditions deep within the Earth and other planets, we use diamond anvil cells and intense lasers to compress and heat mineral samples. The diamond anvils serve as windows for electromagnetic waves allowing us to measure the physical properties of a mineral while it is being compressed and heated. In this way, we can determine the physical properties of different minerals and rocks and compare them with geophysical observations to understand the structure and composition of Earth's deep and otherwise inaccessible interior.
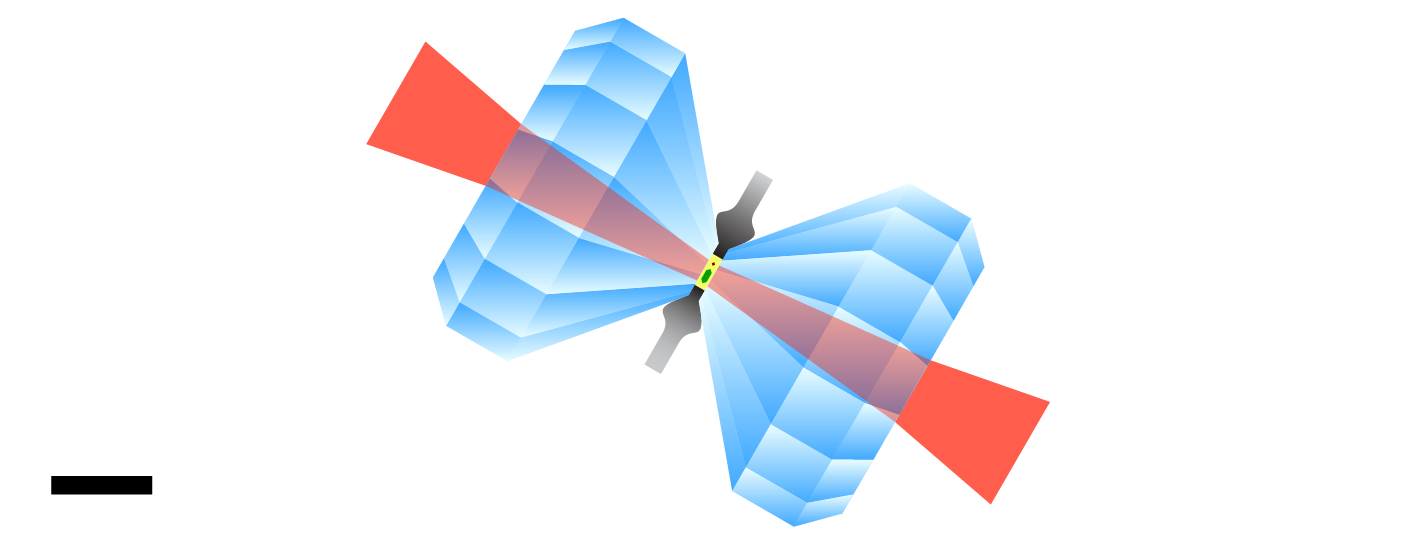
In a diamond anvil cell, two gem-quality diamonds (light blue) are facing each other with their flat-cut tips. The tips confine the high-pressure chamber (yellow) that is sealed to the sides with a thin metallic gasket (grey). The chamber is filled with a soft medium to facilitate (quasi-)hydrostatic compression. A mineral specimen (green) is embedded in the medium and can be subjected to pressures resembling those in Earth's interior by pressing the diamonds together. Pressures in Earth's interior are measured in gigapascal (GPa) and range from 0 GPa at the surface to 364 GPa at the centre of the Earth. A pressure of 100 GPa (1 Mbar) corresponds to one million times the pressure of the atmosphere (1 bar = 14.5 PSI). Infrared lasers (red) can be used to heat the mineral to temperatures of several thousand Kelvin (K) or degrees Celsius (°C); as a reminder T(K) = T(°C) + 273.15.
Structure of the Earth's Interior
Earth's interior can be subdivided into shell-like layers, which are defined by prominent changes in geophysical properties at their boundaries. Changes in density and seismic wave speeds can be detected from the Earth's surface by measurements of the gravitational field, the moment of inertia, tidal deformation, and the characteristics of seismic waves emitted by strong earthquakes. For example, compressional waves (P waves) can propagate through solid and liquid materials while shear waves (S waves) cannot travel through liquids. As a result, the liquid outer core creates a shadow zone for S waves. In general, density and seismic wave speeds are connected to the elastic properties of a material through the compressibility and rigidity of the material. The elastic properties of minerals and rocks can be determined in experiments and compared to geophysical observations. In this way, we seek to understand which materials compose the deep interior of the Earth and how the structure and composition of Earth's interior arose as products of fundamental, persistent processes that are shaping and changing the Earth and other planets since their formation until today.
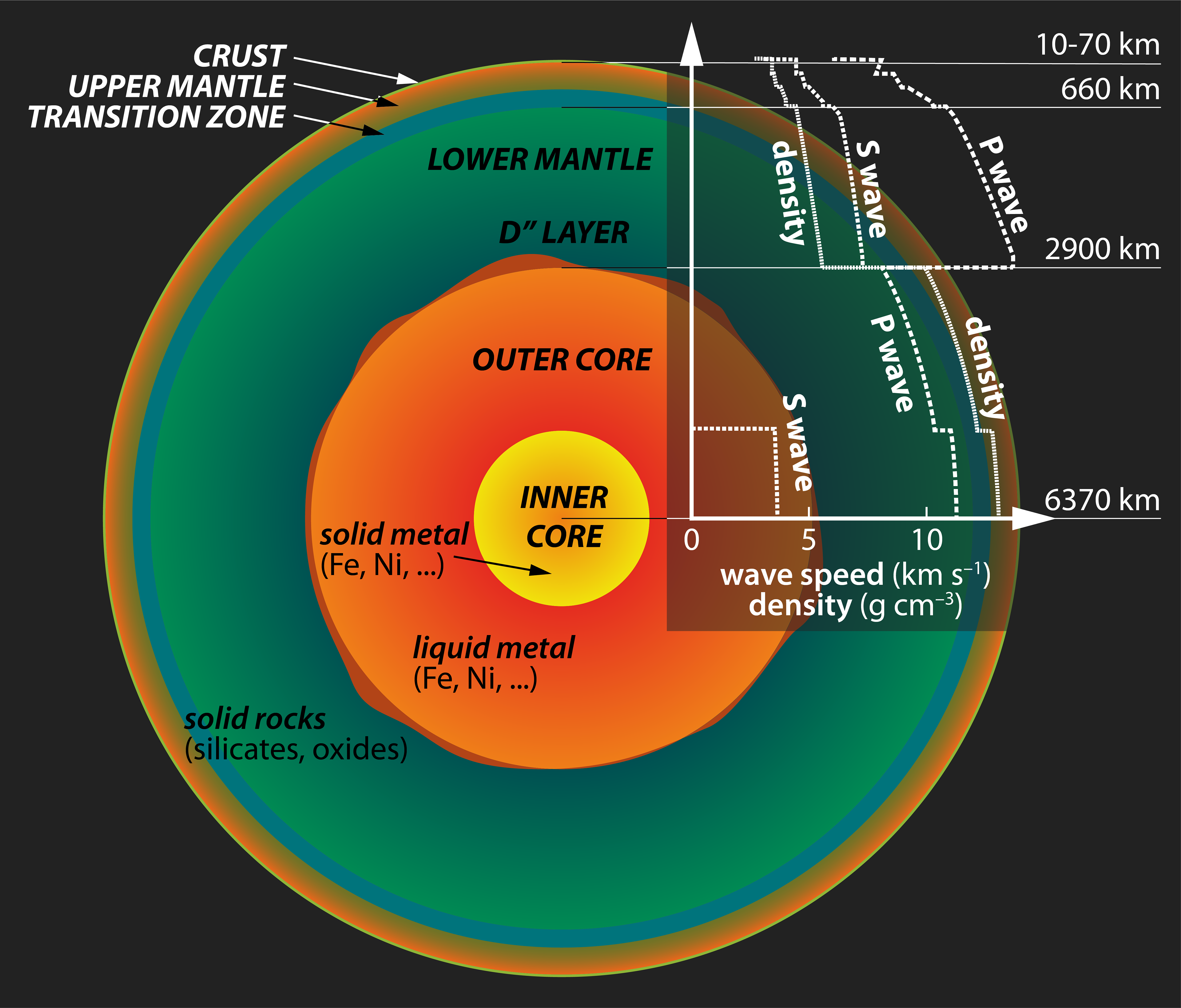
Based on geophysical observations, the Earth can be divided into shells. Earth's thin crust on the surface rests on the mantle, both of wich are composed of predominantly solid rocks. As a result of high pressures and temperatures, the rocks of Earth's mantle can flow very slowly, facilitating the motion of tectonic plates at the surface. Earth's outer core is liquid, and the flow of the liquid iron-nickel mixture generates Earth's magnetic field. The high pressures at the base of the outer core force the iron-nickel liquid to solidify into the iron-nickel alloy of the solid inner core. The diagram in the top-right corner shows the density and the speeds of seismic waves in the different shells. By comparing these geophysical observations with the physical properties of minerals and rocks, we can assess the composition and geological structure of Earth's interior. The graphic above has been constructed from the Preliminary Reference Earth Model (PREM, Dziewonski & Anderson, 1981). PREM represents the overall structure of Earth's interior. Many details of Earth's deep interior, however, remain uncharted.
References
Buchen, J., Marquardt, H., Speziale, S., Kawazoe, T., Boffa Ballaran, T. & Kurnosov, A. (2018) High-pressure single-crystal elasticity of wadsleyite and the seismic signature of water in the shallow transition zone. Earth Planet. Sci. Lett., 498, 77–87.
Dziewonski, A. M. & Anderson, D. L. (1981) Preliminary reference Earth model. Phys. Earth Planet. Inter., 25, 297–356.
Finger, L. W., Hazen, R. M., Zhang, J., Ko, J. & Navrotsky, A. (1993) The effect of Fe on the crystal structure of wadsleyite β-(Mg1–xFex)2SiO4, 0.00 ≤ x ≤ 0.40. Phys. Chem. Minerals, 19, 361–368.
Momma, K. & Izumi, F. (2011) VESTA 3 for three-dimensional visualization of crystal, volumetric and morphology data. J. Appl. Cryst., 44, 1272–1276.
Shatskiy, A., Litasov, K. D., Matsuzaki, T., Shinoda, K., Yamazaki, D., Yoneda, A., Ito, E. & Katsura, T. (2009) Single crystal growth of wadsleyite. Am. Mineral., 94, 1130–1136.
Smyth, J. R. & Hazen, R. M. (1973) The crystal structure of forsterite and hortonolite at several temperatures up to 900°C. Am. Mineral., 58, 588–593.
© Johannes Buchen 2022–2025. Imprint.